Designing for the End: Passive Deorbiting as a Weapon Against Space Debris
Explore a budget-friendly approach to tackling the challenge of space debris
Issue 111. Subscribers 37,466.
As you might have noticed from a couple of our previous posts, we are concerned about the growing problem of space debris. For space exploration to be sustainable, and to prevent the emerging space economy from being crushed by the Kessler Syndrome, we need to act now. We've already covered active deorbiting using low-thrust engines and the challenges of cleaning up defunct satellites and existing debris.
Space Ambition has experienced remarkable growth over the past two years, becoming the largest space tech blog on Substack with 37,000 subscribers and an average of 20,000 views per post. Our audience includes investors, startups, and professionals from the space tech industry.
In 2025, we plan to partner with selected organizations to collaborate on our media initiatives. If you’re interested in exploring this opportunity, please complete the form to start the conversation.
While space has always been a realm of innovation, the presence of over a million pieces of debris larger than 1 cm in orbit demands a shift in focus. Preservation of the space environment must now be considered alongside exploration. With every satellite launched, the risk of space debris collision increases. Designing spacecraft for natural deorbit offers a viable and economical solution, especially for smaller, less expensive satellites.
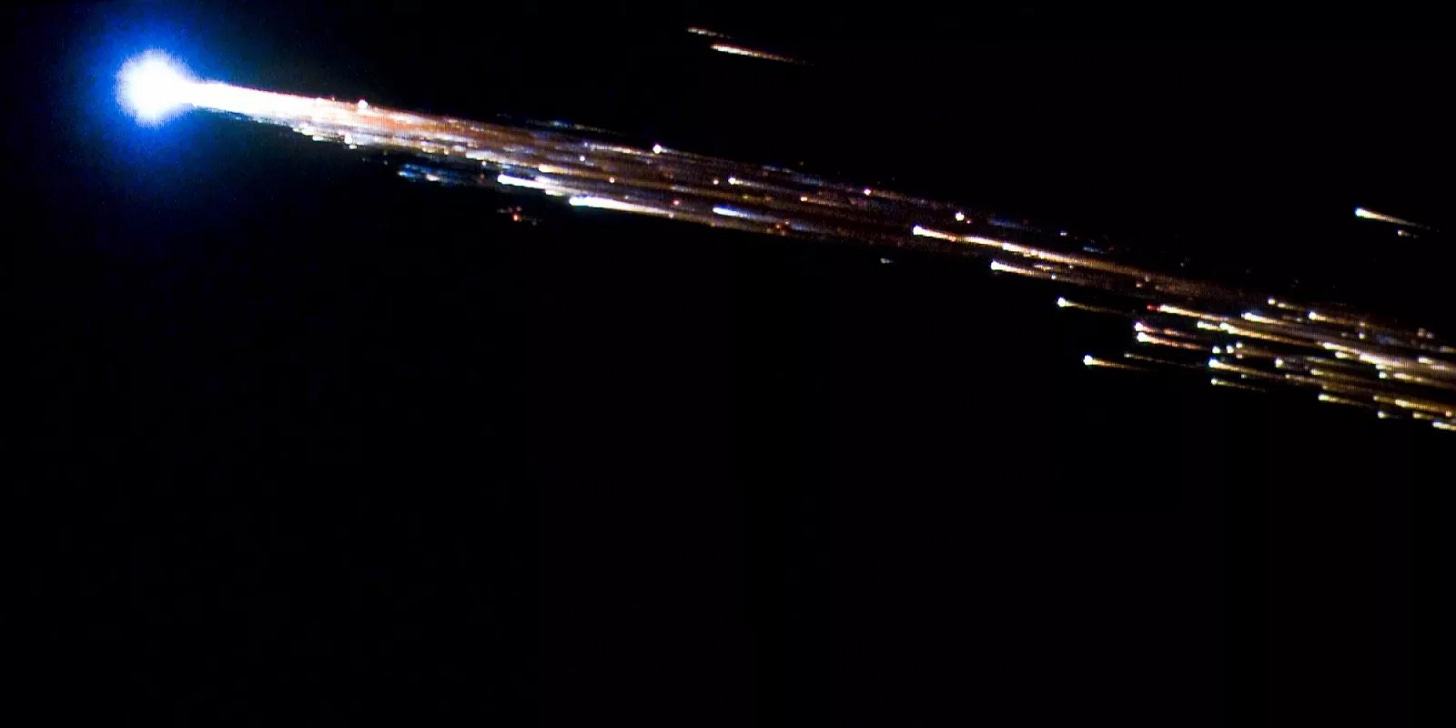
Combating the Debris Problem: A Multi-Layered Approach
There are numerous ways to tackle the debris problem; often, the most effective approach involves combining different methods. Companies like SpaceX frequently employ a multi-layered strategy that incorporates proactive measures, passive mechanisms, and advanced technologies. So let's illustrate this approach with the Starlink constellation as a prime example:
Proactive deorbiting. SpaceX actively deorbits satellites nearing the end of their operational life or those experiencing malfunctions. This controlled descent utilizes the satellite's propulsion system, such as the efficient argon Hall effect thrusters equipped on each Starlink satellite, to lower its altitude and accelerate its re-entry into Earth's atmosphere, where it burns up. These thrusters also enable orbit raising and maneuvering in space for automatic collision avoidance.
Pre-deployment at low altitude. Falcon 9 rockets deploy Starlink satellites at a lower altitude than their operational orbit. This allows engineers to verify the satellites' health and functionality before raising them to their operational altitude, reducing the risk of deploying faulty satellites that could become debris.
Passive deorbiting. Operating in LEO in the range of altitudes between 525 km and 614 km, Starlink satellites experience natural atmospheric drag, which helps to clear any defunct satellites within a few years. This is a crucial design choice for minimizing long-term orbital debris. Passive deorbiting also plays a role in the routine disposal of obsolete satellites. By using Hall thrusters to lower the orbit to around 300 km, atmospheric drag takes over, completing the deorbiting process and reducing the propellant needed for active maneuvers.
In this post, we will delve deeper into the opportunities presented by passive deorbiting.
Harnessing Earth's Atmosphere
Natural deorbit utilizes Earth's atmosphere to gradually slow down and deorbit a spacecraft at the end of its mission. By carefully considering a spacecraft's mass, surface area, and material properties during the design phase, engineers can accelerate this process.
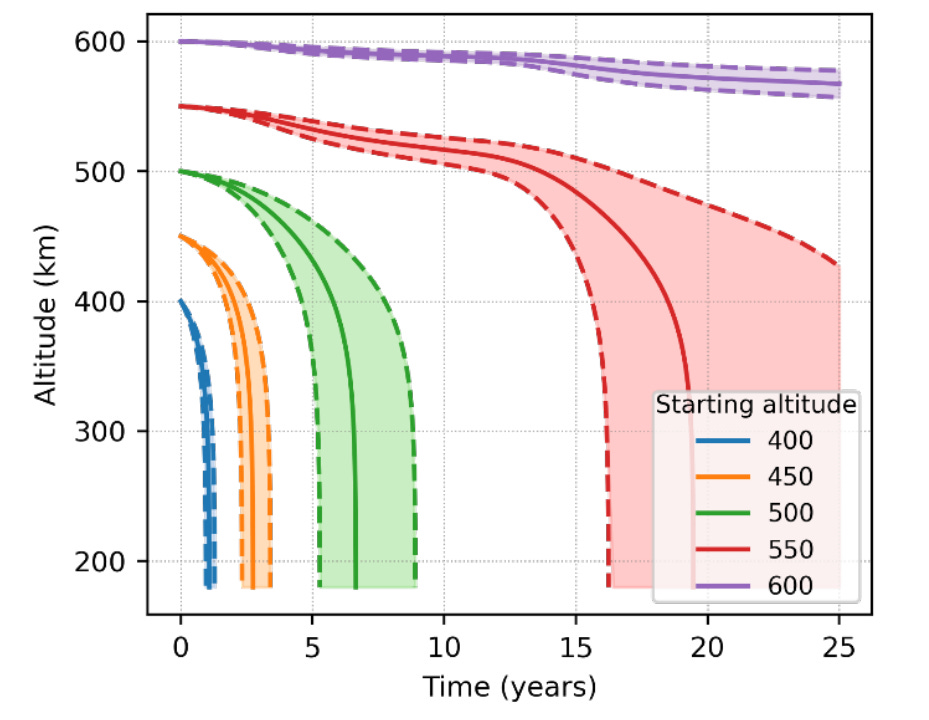
Although space is largely empty, a thin layer of atmosphere persists in LEO (350-2000 km). This causes a drag force on satellites, similar to the resistance you feel when holding your hand out of a moving car window.
The strength of this drag force depends on several factors:
Atmospheric density. Higher altitudes have lower air density, resulting in weaker drag force.
Satellite size and shape. Larger satellites or those with less aerodynamic shapes experience more drag.
Orbital Velocity. Since all objects at a given altitude have roughly the same orbital velocity, we can treat this factor as a constant for our estimation.
We don’t often bother our readers with formulas, but this time we need to demonstrate the calculations so that you better understand the topic. We hope you will like it. To calculate the drag force, we can use the following formula:
Drag = 1/2 * C * ρ * A * V2
Where:
C: Drag coefficient (a dimensionless number of the order of unity, representing the object's shape, often around 2.2)
ρ: Atmospheric density (kg/m³)
A: Satellite's cross-sectional area (in simple terms, part of satellite surface area, exposed to the airflow, m²)
V: Satellite's velocity (approximately 7,700 m/s in LEO)
To illustrate, let's estimate the drag force on a model satellite with a cross-sectional area of 1 square meter, a drag coefficient of 1, and at low solar activity (atmospheric thickness varies with solar weather):
As you can see, the drag force decreases significantly with increasing altitude due to the thinning atmosphere. At 300 km, the drag force is estimated to be 2*10-04 N (200 μN), comparable to the thrust produced by some plasma, electrospray, and even Hall effect thrusters. It's as if the satellite has a permanently active, free deorbiting thruster. At higher solar activity periods, the atmospheric density, and hence, drag can increase up to 200 times.
For unresponsive or non-maneuverable satellites in low orbits (below 600 km), atmospheric drag naturally pulls them back to Earth within 1-5 years. This is considerably faster than the centuries required for satellites at higher altitudes, making it crucial for minimizing long-term orbital debris.
Accelerating Deorbiting
Deorbiting from high-altitude orbits can take years - but is it always true? Are there ways to accelerate natural deorbit or make it feasible at altitudes above 600 km? Ksenia Stebletsova, Co-founder at N42.SPACE sheds light on an intriguing discovery:
When developing one of our services for maneuver analytics, we found some intriguing behavior in certain space objects. Normally, we filter out objects with a perigee [highest orbit point] below 400 km because they naturally slow down and fall due to atmospheric drag. But we saw a similar trend in orbital decay even for objects at much higher altitudes. Why would this happen?
The chart below shows the orbital altitude decay of a piece of debris from the Chinese satellite Fengyun 1C. Over the last 15 days, this fragment dropped around 40 km in altitude. Could this be due to efforts to clean up space debris? Interestingly, this object is categorized in the NORAD catalog as a small item (rcs_size).
After further analysis, we found that these objects have an unusually high drag coefficient (bstar). For example, this particular piece has a drag of 0.04 [in terms of the inverse of the ballistic coefficient, m2/kg], so 100 times higher than the average cubesat. What exactly are these objects? Looking at their size and drag coefficient, we suggest they can be fragments of thermal blankets, or multi-layer insulation (MLI). As the MLI is commonly used in satellites to reflect solar radiation and regulate temperature. You can see an example in the image below, where the Spektr-RG observatory is wrapped in this insulation.
Now the question is how did the MLI detach from its satellite at such a high orbit? Possibly due to degradation over time or collision events! Fortunately, unlike other debris, these fragments are more affected by drag and deorbit quickly, creating a kind of “self-cleaning” effect in Earth’s orbit.
This observation demonstrates the potential for self-cleaning orbits: engineers can optimize spacecraft designs for rapid deorbit. Key design considerations include:
Mass: lower mass reduces the orbital energy needed for a stable orbit, making it easier for atmospheric drag to pull the spacecraft down.
Surface area: a larger surface area increases drag, accelerating deorbit.
Combo: thin films or inflatable structures are more susceptible to atmospheric drag, as they have lower mass and higher surface area.
To maximize a satellite's operational lifespan, passive deorbiting mechanisms can be activated at the end of its mission. Sometimes, these mechanisms, such as sails or inflatable balloons, are integrated directly into the satellite's design by the manufacturer. More recently, third-party companies have begun offering deorbiting services. For example, Vestigo Aerospace provides Spinnaker, a lightweight dragsail that increases atmospheric drag and expedites a satellite's deorbit.
By embracing these simple design principles, we can ensure that space exploration is sustainable. As we venture further into the cosmos, let's commit to preserving the environment both on Earth and in orbit.
In a future article, we plan to explore space debris tracking. Specifically, we're currently discussing the possibility of dual-using radio astronomy infrastructure to track space debris. We welcome your thoughts and insights on this topic at hello@spaceambition.org